Review and comparison of early-stage and commercially available technologies
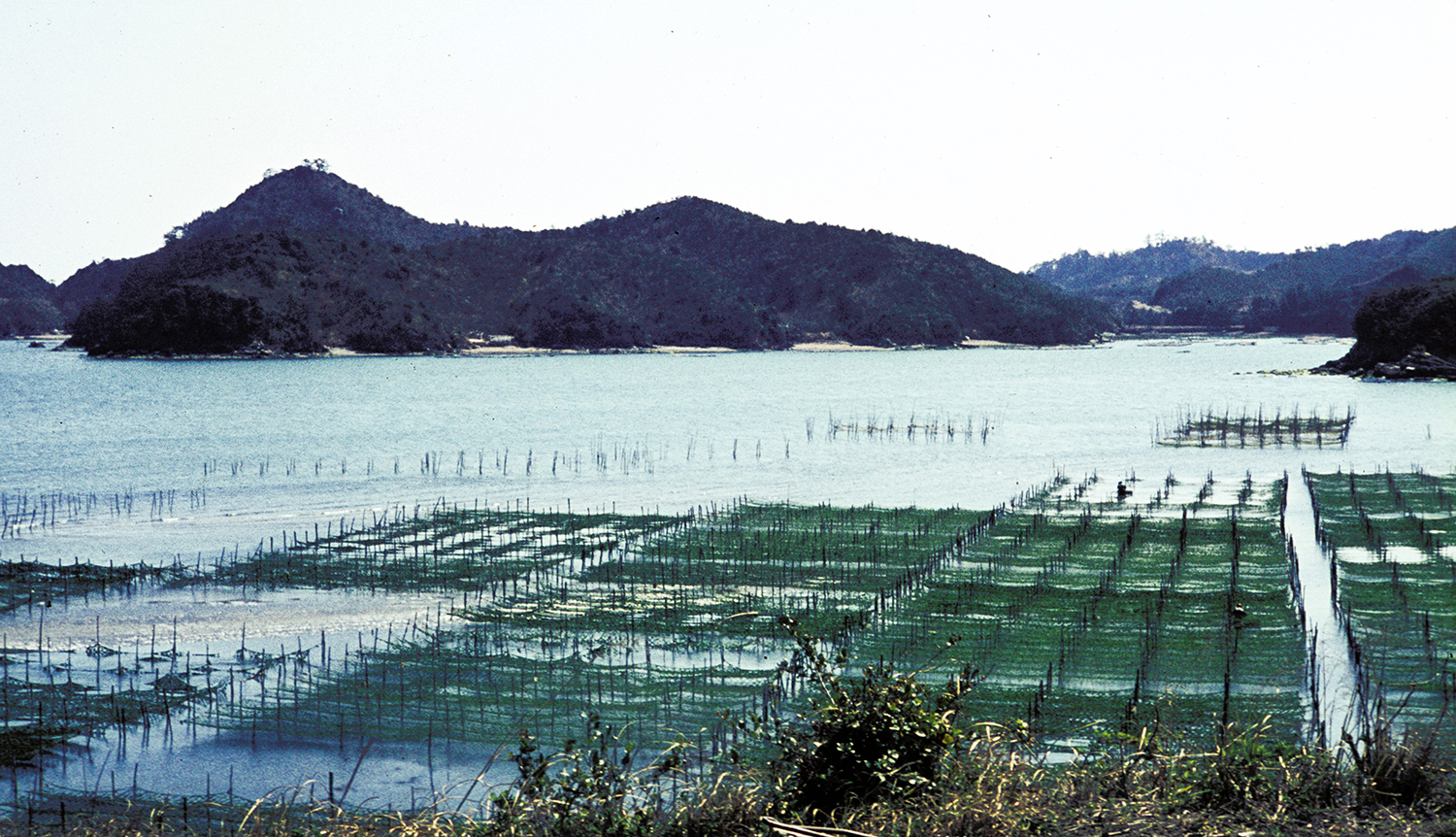
Anthropogenic greenhouse gas (GHG) emissions continue to alter the global climate. As it stands, CO2 contributes the most toward the rise in global temperature when compared to any other GHG. Since the end of the preindustrial age (1750), levels of CO2 in the atmosphere have risen by 47 percent, and, even within just the last decade, levels have risen by 1.4 percent per year on average.
Much of the global society is still reliant on carbon-based fossil fuels, and to keep the rise in global temperature under the 1.5 degrees-C goal set by the Paris Climate Agreement, a transition to carbon-neutral energy sources must be made quickly, but meeting this audacious goal will also require the simultaneous development of negative-emission technologies (NETs) and the implementation of progressive policies, regulations, and incentive structures for rapid technology adoption.
Significant attention and resources have been devoted to terrestrial NETs, with several strategies developed and demonstrated at varying scales, ranging from age-old afforestation/reforestation to hybrid bioenergy with carbon capture and storage (BECCS) and modular direct air capture (DAC) technologies. While the widespread adoption of afforestation and BECCS is limited by competing land-use challenges, the use of DAC is limited due to high cost. Most importantly, while these strategies address CO2 emissions, they fail to tap into the planet’s largest CO2 sink—the oceans.
This article – adapted and summarized from the original publication (Myers, C.R. and V.S. Chinmayee. 2022. Ocean Deacidification Technologies for Marine Aquaculture. J. Mar. Sci. Eng. 2022, 10(4), 523 – reviews and compares early-stage and commercially available technologies for the deacidification of seawater and their suitability for aquaculture.
CO2 in the ocean
The oceans absorb about 33 percent of all anthropogenic CO2 emissions. When compared in equivalent units, bulk CO2 in air and ocean environments is about 720 Gt and 38,400 Gt, respectively. The higher average CO2 concentration and significant CO2 reserves in global oceans offer an opportunity for ocean-based climate solutions. CO2 absorbed by the oceans is converted to carbonic acid and subsequently, H+ and bicarbonate, which decreases the saturation state of calcium carbonate and results in a reduced availability of carbonates for marine organisms to build their shells, leading to decreased productivity and quality of many aquacultured shellfish.
Ocean acidification also has direct impacts on coastal ecosystems, communities and marine-based economies. Hence, ocean-based carbon-capture solutions not only offer opportunities to utilize a concentrated CO2 source for high-impact NETs, but are also a means to address anthropogenic ocean acidification. All the forms of CO2 including bicarbonate (HCO3−) and carbonate ion (CO3-2) are in equilibrium with each other; however, slight changes in pH, temperature, and salinity affect the relative concentrations of those carbon species. Manipulating this carbonate/bicarbonate equilibrium is central to the operation of many ocean CO2 capture technologies.
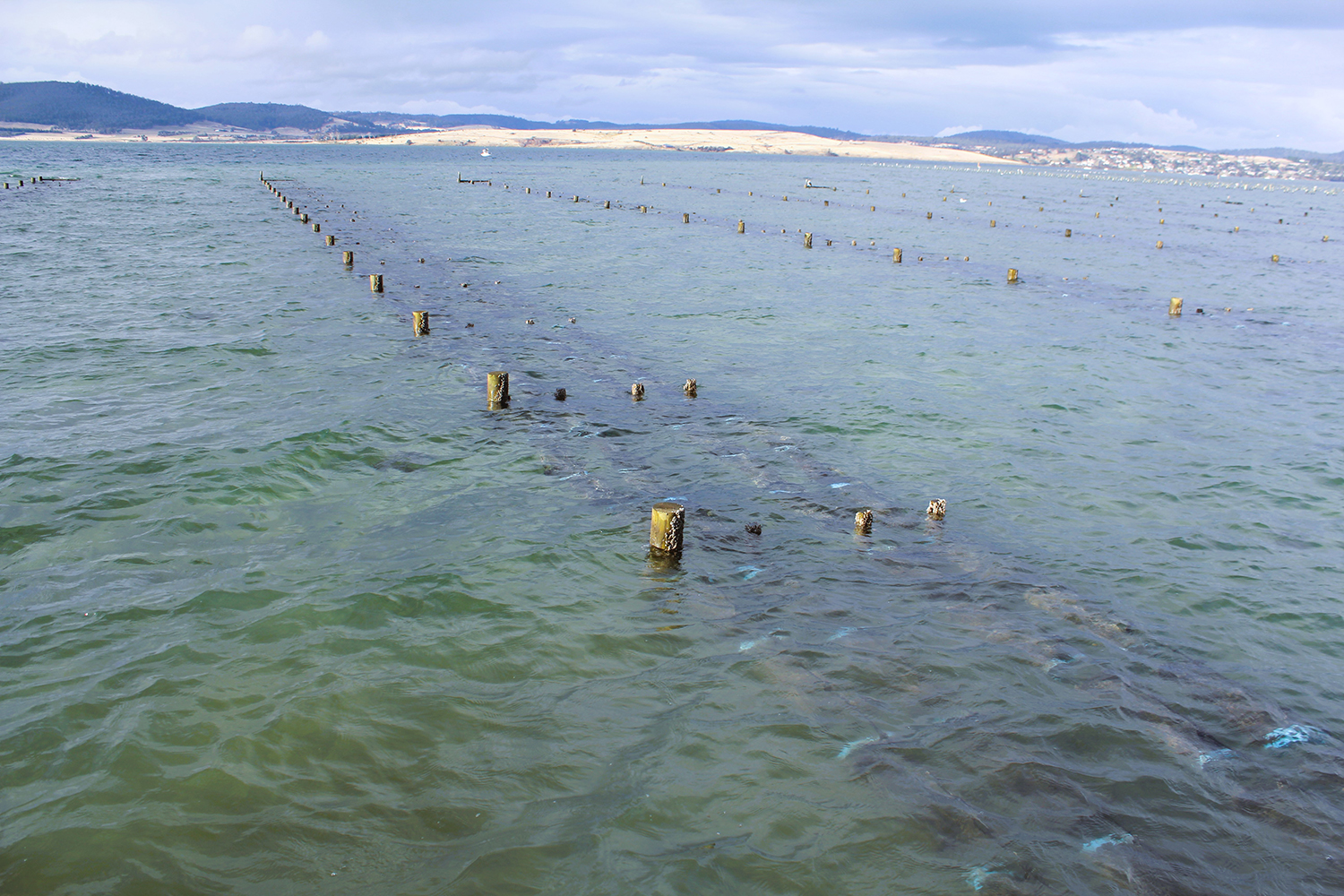
Ocean acidification impacts on marine aquaculture
In addition to the broader impacts of ocean-based CO2 removal, there are direct ecosystem and economic benefits from ocean deacidification. Coastal industries, particularly marine aquaculture, are highly sensitive to ocean CO2 levels and pH changes, which can lower the saturation state of aragonite, a crystalline form of calcium carbonate that many organisms use to build their shells. Shellfish, a general term used to describe aquatic shelled mollusks and crustaceans used for food, are directly impacted by the reduced availability of aragonite to build shells, leading to decreased aquaculture quality and productivity.
Although localized parameters such as ocean current, water temperature, and alkalinity play a role in the rate and extent of acidification, the overall trend of decreasing pH has been seen almost everywhere in the world. Studies have clearly demonstrated the link between CO2 levels and aquaculture quality and productivity. As a result, hatcheries have developed mitigation strategies to minimize uncertainty and to better protect their assets. With consistent monitoring and early detection, localized buffering, site and breeding selectivity, and nutritional enhancements, the aquaculture industry has been able to curtail some of the effects of ocean acidification; however, those methods only offer temporary relief.
Capturing CO2 from the ocean, often referred to as marine carbon dioxide removal (mCDR) could allow for ocean-based climate solutions while simultaneously addressing ocean acidification. Furthermore, when mCDR methods are applied in existing coastal industries such as algal cultivation or coastal infrastructure, a clear economic incentive to deploy ocean-based climate solutions can be established.
Marine carbon dioxide removal methods
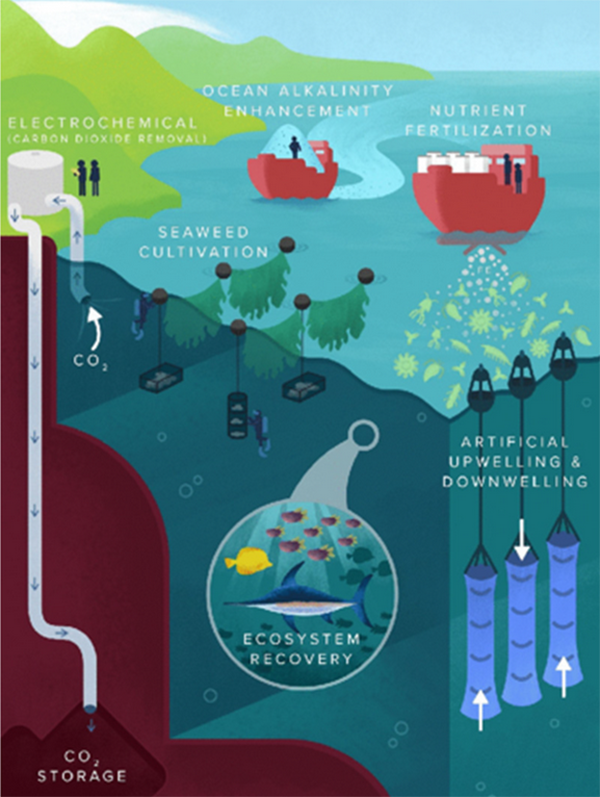
Various mCDR strategies (Fig. 1) have been considered, ranging from artificial upwelling and ocean alkalinity enhancement to seaweed cultivation and electrochemical CO2 stripping. However, given that all the approaches are in the early stages of development and the scarcity field demonstrations to date, their estimated potential for impact is highly variable, and the risks associated with large-scale implementation are not known. The benefits and suitability of these techniques also depend on the local industry (onshore vs. offshore), ecosystem, and competing resource needs.
Given considerations of fish health and food safety, chemical alkalinity enhancement of feedwaters resulting in calcium carbonate (CaCO3) precipitation (i.e., calcium depletion) seems nonideal. On the other hand, for seaweed cultivation, despite being a chemical input-free carbon capture strategy, the spatiotemporal pH changes generated are not known. Furthermore, aquaculture facilities may need additional resources to grow and manage seaweed, making it less attractive. Hence, several promising mCDR strategies may not be suitable for the deacidification of aquaculture feedwaters.
Specific to marine aquaculture, which is expected to significantly grow by 2050, coupling with modular mCDR systems that can be easily added to existing seawater intake at the facilities would be preferable. Additionally, systems that can be operated using renewable energy and with minimal chemical inputs would allow for sustainable seafood production. Here we review technologies suitable for deacidifying feedwaters for coastal aquaculture.
Technologies for CO2 removal from aquaculture feedwaters
There are CO2 removal methods suitable for the deacidification of marine aquaculture feedwaters, both commercially available technologies and methods in the early stages of development. These methods can be broadly classified into physical (trickling filter), chemical (ion exchange), and electrochemical (bipolar membrane electrodialysis and electrolytic cation exchange) approaches.
Trickling filter and recirculation aquaculture systems
Recirculating aquaculture systems (RAS) involves growing fish in a closed-loop set of tanks where water is recycled, and the addition/removal of nutrients/toxins is heavily controlled by different types of filters. As a result, the oxygen concentration in the water decreases over time due to respiration, while CO2 and nitrogen concentrations increase. Elevated levels of CO2 in the water are highly toxic to fish; thus, its cost-effective removal is very important.
While RAS offer a relatively simple physical method for the reduction of dissolved CO2 in water, they only remove CO2 from water when the relative concentration is higher in the water than in the surrounding air. Albeit simple and commercially available, the implementation of large-scale TF and RAS to remove CO2 from ocean water will also require subsequent CO2 capture strategies in order to be environmentally sustainable.
Ion-exchange resins
Ion-exchange resins (IERs) are widely used in water treatment and in industrial processes. The resins are sold as anion- and cation-exchange types. Both resin types need to be regenerated upon saturation using chemical solutions (acid, base, salt solution). IERs for carbon capture may be an option in the future if electrical resin regeneration either directly via water splitting or indirectly via electrochemical onsite acid/base generation from seawater and renewable energy is used. Given the limited promise of IER at this stage, the method is not discussed further in this review.
Bipolar membrane electrodialysis
A bipolar membrane electrodialysis (BPMED) unit is made up of multiple membrane layers stacked between an anode and cathode. When adequate energy is supplied to the unit, water at the surface of the bipolar membrane (an ion-exchange membrane made up of a cation- and an anion-exchange layer) dissociates into hydrogen and hydroxide ions. The movement of the ions is dictated by the applied potential, causing cations to move in the direction of the cathode and anions to move toward the anode.
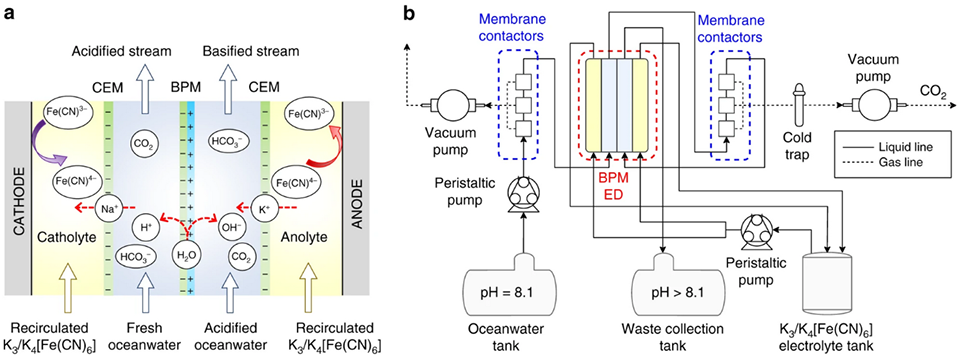
Various BPMED prototypes have been designed and tested using synthetic seawater, operating on the same fundamental principles but differing in membrane configuration and electrolyte solutions, leading to different energy efficiencies for CO2 removal.
Although BPMED offers an elegant solution to ocean deacidification, the technology is in its very early stages. The pathway has not yet been tested in an ocean environment; instead, only synthetic seawater and brine solutions in the lab have been used. Thus, the technology performance in harsh marine conditions is unknown. Both methods rely heavily on membranes which are expensive and highly susceptible to fouling/scaling.
Electrolytic cation exchange
The electrolytic cation exchange module (E-CEM) was designed primarily to generate fuels from seawater for naval applications. E-CEMs have been tested in different configurations involving the presence/type of ion exchange resin in the compartment, the electrode compartment volume, and the endplate material. E-CEM technology in its current configuration is not optimized for CO2 capture from seawater, and if CO2 generation is the ultimate goal, decoupling the process from hydrogen and oxygen production may improve overall process efficiency.
Comparison of technologies
Technologies for CO2 removal from seawater are in their early stages of development, with only one technology being successfully demonstrated in field settings. As a result, it is important to note the benefits and drawbacks of the different approaches to recognize how they can be tailored for marine aquaculture (Table 1).
Myers, Deacidification, Table 1
CO2 Removal Method | Feedwater Used | Flow Rate | CO2 Capture Efficiency | Product |
---|
CO2 Removal Method | Feedwater Used | Flow Rate | CO2 Capture Efficiency | Product |
---|---|---|---|---|
Trickling filter (TF ) in RAS | Freshwater, seawater | 100 L/min | CO not captured | CO2 |
BPMED (via acidification) | Synthetic seawater, brine | 2100 mL/min | 80.80% | CO2 |
BPMED (via basification) | Synthetic seawater | Not reported | Not reported | CaCO3 |
BPMED (via acidification) | Synthetic seawater | 37 mL/min | 71% | CO2 |
BPMED (with reactive crystallization) | Synthetic seawater | 667 mL/min | 50% | CaCO3 |
E-CEM | Natural seawater | 1900 mL/min | 92% | CO2, H2 |
Perspectives
RAS technology has already demonstrated CO2 removal from fresh and seawater systems, and it is the most cost-effective deacidification approach for aquaculture; however, it is not designed to capture CO2. The energy consumption by RAS operations is high relative to other methods, which is most likely caused by the need for temperature equilibration after evaporative cooling within the trickling filter. Adding a membrane contactor would enable CO2 capture and make RASs more sustainable, but membrane contactors are expensive and add energy and maintenance costs. It should be noted that the other methods included in Table 1 either do not integrate pumping costs into the overall energy calculations or assume colocation with a desalination plant to minimize pumping-related capital and operating expenses. Hence, a direct comparison of RAS energy use to that of the other (emerging) technologies may not be very meaningful.
Although achieved in different ways, electrochemical methods rely on a pH swing to release CO2 by altering the carbonate/bicarbonate equilibrium. If operated using renewable energy, BPMED can offer a sustainable solution to deacidification with CO2 capture, by producing either gaseous CO2 (acidified approach) or solid CaCO3 (basified approach). The CO2 produced can be used as a precursor for chemicals or as a feedstock for algae growth. While capture via CaCO3 precipitation produces an easy-to-handle solid product and eliminates the need for expensive membrane contactors, the process decreases calcium availability in feedwater, thus negatively impacting aquaculture quality and productivity.
Current mCDR technologies that are suitable for the deacidification of aquaculture feedwaters are in their very early stages of development and must improve energy efficiency, enhance material durability in harsh marine conditions, and decrease component costs to be viable for aquaculture applications. The lack of specialized infrastructure and the difficulty in accessing reliable and inexpensive sources of carbon-neutral energy are additional considerations.
Although the technologies are in their early stages of development, their modular design and distributed deployment potential offer a promising outlook. However, significant research and development are required to take these technologies from lab to market, and given the pressing need for sustainable deacidification solutions, making the systems cost-effective in the near term may depend on the availability of government subsidies or tax credits.
Now that you've reached the end of the article ...
… please consider supporting GSA’s mission to advance responsible seafood practices through education, advocacy and third-party assurances. The Advocate aims to document the evolution of responsible seafood practices and share the expansive knowledge of our vast network of contributors.
By becoming a Global Seafood Alliance member, you’re ensuring that all of the pre-competitive work we do through member benefits, resources and events can continue. Individual membership costs just $50 a year.
Not a GSA member? Join us.
Authors
-
Christopher R. Myers
Corresponding author
Energy and Environment Directorate, Pacific Northwest National Laboratory, Sequim, WA 98382, USA -
Chinmayee V. Subban, Ph.D.
Energy and Environment Directorate, Pacific Northwest National Laboratory, Seattle, WA 98109 USA; and Department of Materials Science and Engineering, University of Washington, Seattle, WA 98195 USA
Tagged With
Related Posts
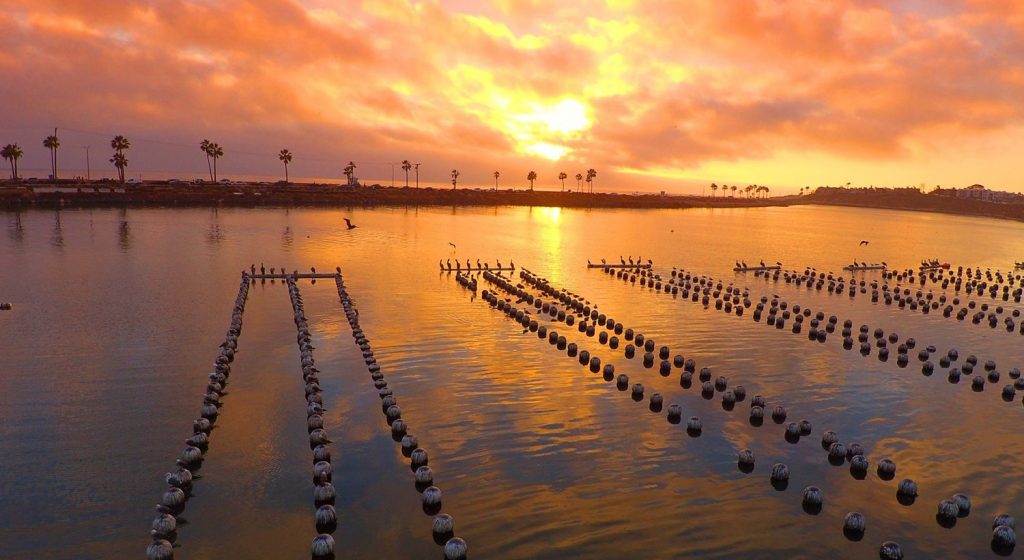
Responsibility
As ocean acidification threatens the shellfish industry, this California oyster farm is raising oysters resistant to climate change
Despite the dangers to shellfish posed by ocean acidification, a forward-thinking California oyster farm is producing oysters resistant to climate change.
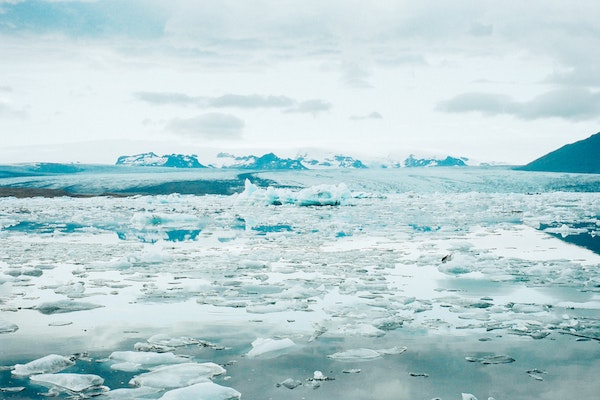
Responsibility
Fast-melting Arctic ice may contribute to an ‘extraordinary increase’ in ocean acidification
A study has found an “extraordinary increase” in ocean acidification and a strong correlation with the increasing rate of Arctic ice melting.
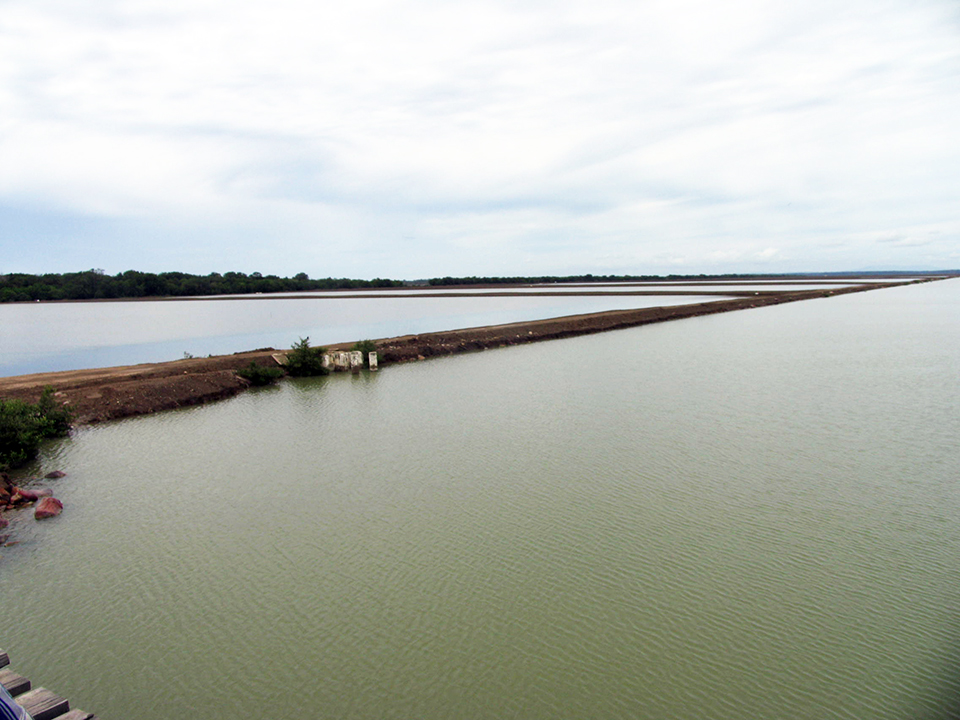
Responsibility
Atmospheric pollution affects water quality
Acid rain typically does not heavily affect aquaculture operations, and application of agricultural limestone can buffer water against the impacts of acid rain at facilities that use stream water.
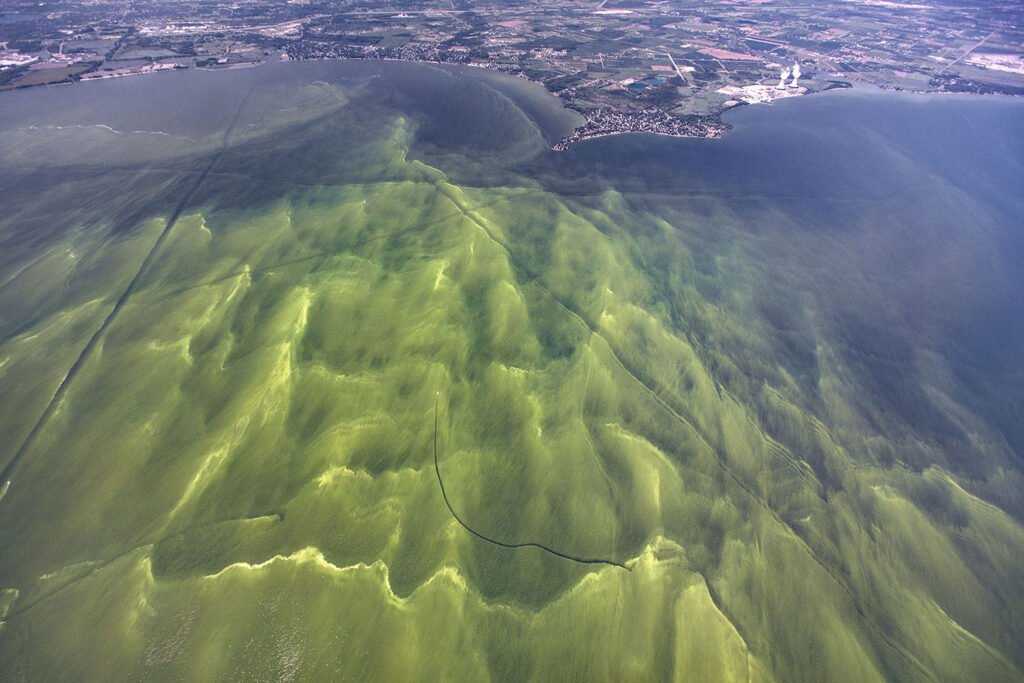
Responsibility
Climate change effects on aquaculture production
Comprehensive review explores the negative and positive sides of climate change on aquaculture production, and implications for its sustainability.